Engineered Covers for Waste Containment
Monitoring Engineered Covers for Waste Containment
Robert C. Reedy and Bridget Scanlon (February 2003)
The growing realization that remediation of many contaminated sites is technically infeasible has resulted in a shift in emphasis to containment as an alternative to remediation. Monitoring is required to demonstrate the effectiveness of engineered cover systems in minimizing infiltration into underlying waste. The purpose of this study is to evaluate a variety of monitoring technologies. Monitoring systems were installed in a resistive (GCL/asphalt) barrier at 1.3 m depth and a conductive (capillary) barrier at 2.0 m depth constructed near El Paso, Texas, in 1997. Monitoring of most systems began in October 1997. The monitored components of the water balance include precipitation, surface runoff, lateral drainage from the asphalt layer, deep drainage, and water content. Evapotranspiration (ET) is calculated as the residual of the monitored components. Ground conductivity measurements using an EM38 (Geonics, Mississauga, Ontario) in the vertical and horizontal dipole modes were conducted concurrent with the neutron probe measurements to evaluate electromagnetic induction (EM) as a non-invasive technique for monitoring water content. Results of the water balance monitoring for the last three months of 1997 and for the complete years 1998 through 2000 are presented in Table 1. Net infiltration was calculated as the sum of precipitation and irrigation minus runoff. The storage values shown are the results of the neutron probe surveys. The data indicate that both barrier designs have performed similarly. However, annual precipitation has averaged only 56% to 75% of the 30-year average (116% for 1998 including irrigation) with the result that neither barrier has been sufficiently stressed to induce drainage.
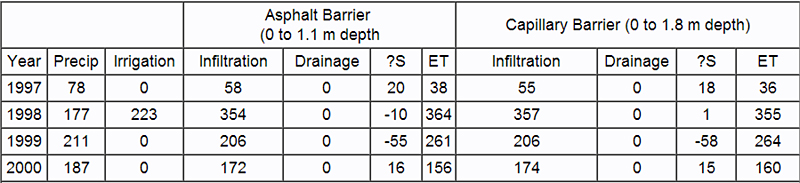
Table 1. Water balance parameters (mm) for specified depth intervals of the two barrier designs.
TDR has been of limited use for monitoring water content at our site. Periods of higher water content and/or higher soil temperature increased the sandy clay loam subsoil bulk conductivity to the point that TDR waveforms displayed little or no reflections. Probes installed in the topsoil were generally less affected. Water content values derived from analysis of the less attenuated waveforms were not in agreement with the neutron probe measurements despite calibration of the TDR probes using site soils. The TDR measurements also displayed seasonal changes in water content ranging from 0.05 to 0.10 m3/m3 at depths where no changes were indicated by the neutron probe measurements.
Electromagnetic induction has proved to be a rapid and accurate method for determining water storage at our site. The models predict water contents to within 0.007 m3/m3 of the measured averages. The GCL/asphalt barrier models had similar results. Using each of the individual location models to calibrate all of the remaining location data resulted in predicted water contents averaging within 0.015 m3/m3 of the measured average. These results demonstrate the usefulness of applying electromagnetic induction to obtain accurate measurements of water storage.
Heat dissipation sensors have been more reliable than thermocouple psychrometers for monitoring soil water potential at our site. The HDS instruments displayed rapid and stable responses to changes in water content and the data are in agreement with the neutron probe measurements. Duplicate HDS instruments generally displayed measurements within 10% of each other at potential values above -1.0 MPa. In contrast, most of the TCP data were not in agreement with the HDS or neutron probe data and duplicate TCP instruments rarely displayed similar responses. Heat dissipation sensors provide accurate measurements in the wet range (=-0.5 MPa) and have the additional advantage of measuring a wider range of potential energy values (-0.01 to -1.0 MPa) compared to thermocouple psychrometers (-0.5 to -8 MPa).
Reference
Reedy, R. C., and Scanlon, B. R., 2002, Long-term water balance monitoring of engineered covers for waste containment, in 2001 International Containment and Remediation Technology Conference, Orlando, Florida, Institute for International Cooperative Environmental Research, Florida State University, Paper ID. No. 073, http://www.iicer.fsu.edu, 3 p. [PDF]
Modeling of Engineered Covers for Waste Containment
Bridget Scanlon, Principal Investigator; Robert C. Reedy (February 2003)
The purpose of this study was to compare water-balance simulation results from 7 different codes, HELP, HYDRUS-1D, SHAW, SoilCover, SWIM, UNSAT-H, and VS2DTI, using 1-3 yr water-balance monitoring data from nonvegetated engineered covers (3 m deep) in warm (Texas) and cold (Idaho) desert regions. The codes evaluated in this study are listed in Table 1. Graphical user interfaces are available for most codes. SHAW has an interface for data input but does not have a postprocessor. Detailed descriptions of the codes can be found in the user's manuals. It is impossible to describe all the attributes of the various codes in this paper; however, many of the attributes related to water-balance modeling for HELP, HYDRUS, SoilCover, and SHAW are described in Wilson et al. (1999).
HELP (Hydrologic Evaluation of Landfill Performance, Version 3; Schroeder et al., 1994): |
HYDRUS1D (Version 3.0; Simunek et al., 1998) |
SHAW (Simulation of Heat and Water; Version 2.4; Flerchinger and Saxton, 1989; Flerchinger et al., 1996) |
SoilCover (Version 4.1; Wilson, 1990; Wilson et al., 1994; GEO2000, 1997) |
SWIM (Soil Water Infiltration and Movement model; Version 2; Verburg et al., 1996):http://www.clw.csiro.au/products/swim |
UNSAT-H (Version 3.0; Fayer, 2000) |
VS2DTI (Variably Saturated 2 Dimensional Transport Interface; Version 1; Healy, 1990):http://water.usgs.gov/software/vs2di.html |
Sites
The Texas site is located near Sierra Blanca, which is about 120 km southeast of El Paso, Texas. The site is within the Chihuahuan Desert of Texas. Long-term (30-yr) mean annual precipitation at Sierra Blanca is 320 mm. The site consists of heavily instrumented engineered covers that were installed in the summer of 1997. The surface dimensions of the engineered cover are 34 × 17 m.
The Idaho site is located at the Idaho National Engineering and Environmental Laboratory in southeastern Idaho (Porro, 2001) on the Snake River Plain. Long-term (40-yr) mean annual precipitation is 221 mm. The site consists of a concrete structure containing 10 cells, each of which is 3 m × 3 m × 3 m (four walls and a floor). Replicates of a monolithic soil cover and a capillary barrier cover were constructed in the cells. Data from only one of the monolithic soil cover cells are used in this study.
Codes
This study demonstrates the variability in simulated water-balance components using a variety of codes (listed above in Table 1) on the basis of field monitored data from engineered covers at warm (Texas) and cold desert (Idaho) sites and provides some indication of the expected reliability of simulated water balances. Simulation results from most codes were similar and generally reproduced measured water-balance components at the Texas and Idaho sites. Both sites consisted of unvegetated loam soil.
Simulation of infiltration-excess runoff was a problem for all codes, underscoring the difficulties of representing actual precipitation intensities and of measuring hydraulic conductivity of surficial sediments (as shown by the data from Texas). Drainage is the most critical parameter for evaluation of contaminant transport, engineered covers for waste containment, and groundwater recharge. Drainage could be estimated to within ~ ± 64% by most codes. Outliers for the various simulations could be attributed to the following factors:
- the modeling approach, i.e., water-storage routing versus Richards' equation,
- the upper boundary condition during precipitation and time discretization of precipitation input,
- water retention functions (i.e., van Genuchten versus Brooks and Corey), and
- the lower boundary condition (i.e., unit gradient versus seepage face).
The water storage routing approach does not seem to adequately represent the flow system in semiarid regions. By assuming that gravity is the only driving force and ignoring matric-potential gradients that are often upward in semiarid regions, downward flow is generally overestimated and ultimately results in overestimation of drainage.
The approach used to simulate the upper boundary condition during precipitation is crucial when precipitation is input on a daily or larger time step. Setting PE to zero on rain days (VS2DTI) greatly underestimated evaporation and overestimated drainage. Subtracting PE from precipitation and applying net precipitation or net PE on a daily basis (HYDRUS-1D) had a much lesser impact on simulation results. The best approach is to disaggregate daily precipitation and apply it at a specified rate, allowing PE to occur throughout the rest of the day, as shown by the UNSAT-H simulations.
The impact of water retention functions was demonstrated at the Idaho site, where increased unsaturated hydraulic conductivity based on the Brooks and Corey functions relative to the van Genuchten functions resulted in overestimation of evaporation and underestimation of drainage. In contrast, the input value of residual water content (0 for Campbell function versus > 0 for Brooks and Corey) had little impact on simulation results.
The most appropriate lower boundary condition for simulating wickless lysimeters is a seepage face. Simulations using HYDRUS-1D demonstrated that this boundary condition could be approximated by simulating a thin bottom layer of gravel with a unit gradient boundary condition in codes that use Richards' equation but do not include a seepage face option. However, use of a unit-gradient lower boundary condition alone greatly overestimated drainage. This study demonstrates the usefulness of conducting intercode comparisons to evaluate the reliability of water-balance simulations and to determine important factors controlling water-balance simulation results.
References
Scanlon, B. R., Christman, M., Reedy, R. C., and Gross, Beth, 2002, Intercode comparisons for simulating water balance in an engineered cover, in 2001 International Containment and Remediation Technology Conference, Orlando, Florida, Institute for International Cooperative Environmental Research, Florida State University, Paper ID. No. 148, http://www.iicer.fsu.edu, 3 p. [PDF]
Scanlon, B. R., Christman, M., Reedy, R. C., Porro, I., Simunek, J., and Flerchinger, G. N., in press, Intercode comparisons for simulating water balance of surficial sediments in semiarid regions: Water Resources Research. [PDF]
Scanlon, B. R., Christman, M., Simunek, J., and Reedy, R. C., 2001, Intercode comparisons for simulating water balance of near-surface soils (abs.), in Eos, v. 82, no. 47, Fall Meeting Supplement, American Geophysical Union, Abstract H12F-10.[PDF]